The risk factors associated with the development of Metabolic Syndrome (MetS) are similar to those associated with hypertension, diabetes and obesity. It is important to note that additional variables such as lifestyle, sex, ethnicity and genomic predisposition also impact the development of the syndrome. The complex nature of MetS has made it difficult for researchers to identify the specific genetic causes. Different genomic and molecular approaches have been applied to understand the trait’s genetic basis. The results of these studies suggest that MetS is a polygenic condition, which means that it is influenced by multiple genes, while environmental factors also play a role.
Therefore, the inheritance of a single specific genetic variant, or allele, may be less important than the combined, additive effects of inheriting many of them. Such combinations of genetic variants together can influence traits or disease risks. However, the current understanding of MetS suggests that certain risk alleles relevant to the individual components of the disease may also have an overlapping role, contributing to the overall risk of developing Metabolic Syndrome.
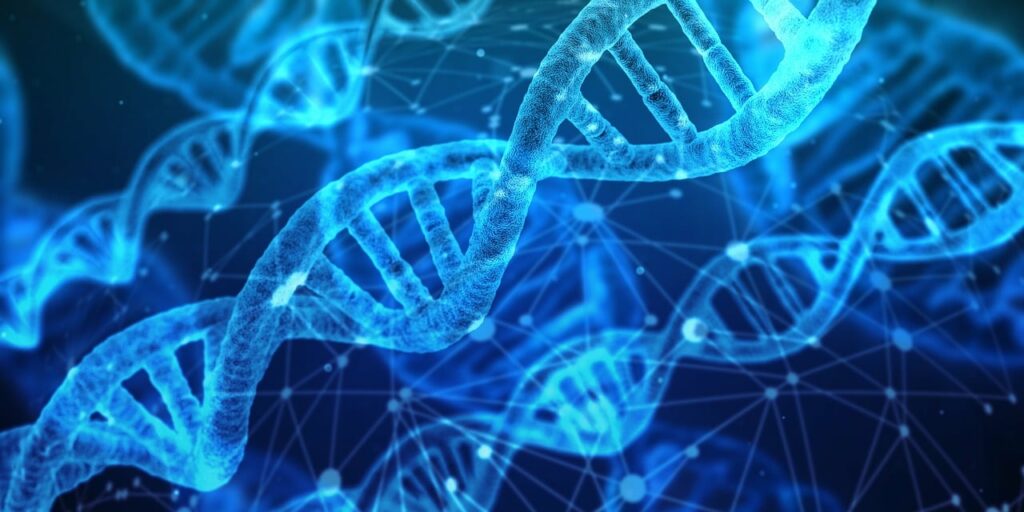
Deciphering the MetS Code: A Multi-faceted Approach
MetS is a complex health condition involving several key factors, including altered blood cholesterol profiles, blood pressure, obesity and insulin resistance. These factors significantly increase the risk of developing cardiovascular disease and type 2 diabetes, representing a significant contributor to the healthcare burden (1, 2). It has been demonstrated that genetic factors are significantly associated with the disease components and contribute to the risk of developing MetS (3-6).
Gene alterations, or mutations, are changes in the DNA sequence of a gene. The effects of such genetic changes can vary greatly. In some cases, they may have no noticeable effect, while in others they may lead to health issues. Genes can be investigated using a variety of approaches and techniques, including the analysis of individual or combinations of genes linked to MetS. Among them, we can find:
–Linkage analysis, a technique used to identify the locations of genes that may be associated with particular diseases or traits within families.
–Genome-wide association studies (GWAS), aim to identify common genetic variants in large populations associated with particular diseases or traits. These involve scanning the genomes of thousands (or even millions) of people to identify single nucleotide polymorphisms (SNPs) – tiny genetic variations – more common in people with the trait or disease. By comparing the frequency of SNPs in “positive” cases -people with the trait- and controls -people without the trait- researchers can identify genetic factors associated with the trait.
-Studying epigenetic modifications, regulations that can be inherited and that make genes more or less active without changing the genetic code. These involve chemical modifications of DNA that can be influenced by environmental factors (such as diet, stress and toxins).
–Proteomics, that addresses the large-scale study of proteins, which are the functional molecules that carry out most cellular processes.
-Studying microRNAs, a series of small non-coding RNAs that play an important role in regulating gene expression – the process by which the information stored in a gene is used to make a functional protein.
The combination of approaches like all the previous provides powerful means for identifying the genetic factors that predispose individuals to MetS.
The Genetic Blueprint of Metabolic Syndrome: A Complex Tapestry
The two most significant risk factors for cardiovascular disease in patients with MetS are dyslipidemia and hypertension. Both of these conditions result from a combination of genetic and environmental factors (and their interplay) and therefore are considered polygenic conditions.
Fluctuations in blood lipids increase the likelihood of developing MetS (7). Familial hypercholesterolaemia is a common genetic cause of the alteration of blood lipid profiles, or dyslipidaemia, even if dietary intake also plays a role (8). This disorder, which affects 1 in 500 individuals, elevates LDL levels and involves more than 700 distinct mutations in LDL receptor genes (9).
Other lipoprotein disorders are characterised by high triglycerides and low HDL cholesterol. Most cases have mutations in the LPL and APOE genes, important for lipoprotein metabolism. APOE4 is most strongly associated with vascular inflammation and cardiovascular disease. Mutations in these genes contribute to an increased risk of myocardial infarction, a component of MetS (10). To date, more than 50 genes have been identified as being significantly associated with blood pressure and/or hypertension (11).
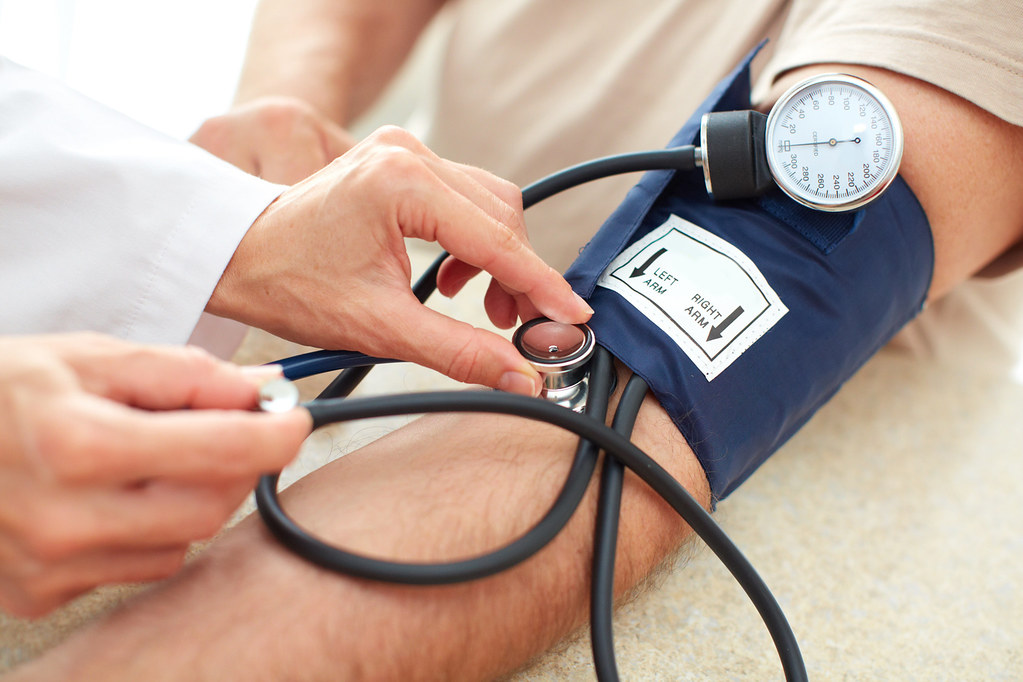
Individuals with a family history of hypertension are at a greater risk of developing MetS than those with secondary hypertension. These relatively uncommon syndromic disorders of blood pressure regulation typically follow Mendelian inheritance patterns with high penetrance, indicating that a person with the allele in question is also likely to have the disease. The genes responsible for these disorders create proteins that can affect renal electrolytes and water handling (12). This can occur indirectly by modifying the expression of adrenal/mineralocorticoid hormones, or directly by influencing the function of renal sodium transporters (12).
Excess body weight contributes to the development of MetS and increases the risk of developing cardiovascular, renal and diabetes complications. Obesity may act as a genetic trigger, activating the interactions between genes responsible for MetS components (13). In particular, some studies have shown that the genetic basis of MetS depends on fat distribution. In this context, central fat distribution (fat around the abdomen) indicates a higher risk of developing MetS (14, 15). The process of elucidating the risk alleles for obesity is complex, and the research methodologies used to collect and manage specimens, as well as to extract genetic material, are still being refined (16).
Two of the primary genes associated with obesity are the melanocortin receptor 4 (MC4R) – a membrane receptor that mediates hormonal signals from adipose tissue – and the fat mass and obesity-associated gene (FTO). The first has been proven to be linked to fat accumulation, while the other is more directly associated with the development of MetS. MC4R has been most commonly associated with monogenic forms of obesity, such as in human MC4R deficiency mutations (17). However, it may also play a role in polygenic forms of common central obesity. Although its physiological function is not yet fully understood, the FTO gene was one of the first to be associated with BMI (18). This specific SNP has also been identified as more prevalent in individuals with MetS (19).
Type 2 diabetes (T2D) has been shown to have a higher prevalence in certain ethnic groups, providing evidence for a genetic predisposition to this complex disease. This is evidenced by differences in allele frequencies of predisposing genetic polymorphisms. Some studies have indicated that the genetics of T2D and MetS may have some influence from overlapping genes. However, the individual effect sizes – the strength of a relationship between two variables – of each genetic variant are relatively small (20).
It has been demonstrated that variants of the TCF7L2 gene are particularly associated with an elevated risk of developing both T2D and MetS (21). TCF7L2 may contribute to the development of MetS by causing excess fat and glucogen deposition in the liver and hyperlipidaemia, glucose intolerance and, eventually, T2D (22). Given the relatively minor impact of individual SNPs, it is postulated that an individual’s susceptibility to MetS is not solely attributable to a single variant. Rather, these variants interact with one another, creating a synergistic effect that contributes to the development of both MetS and T2D (22).
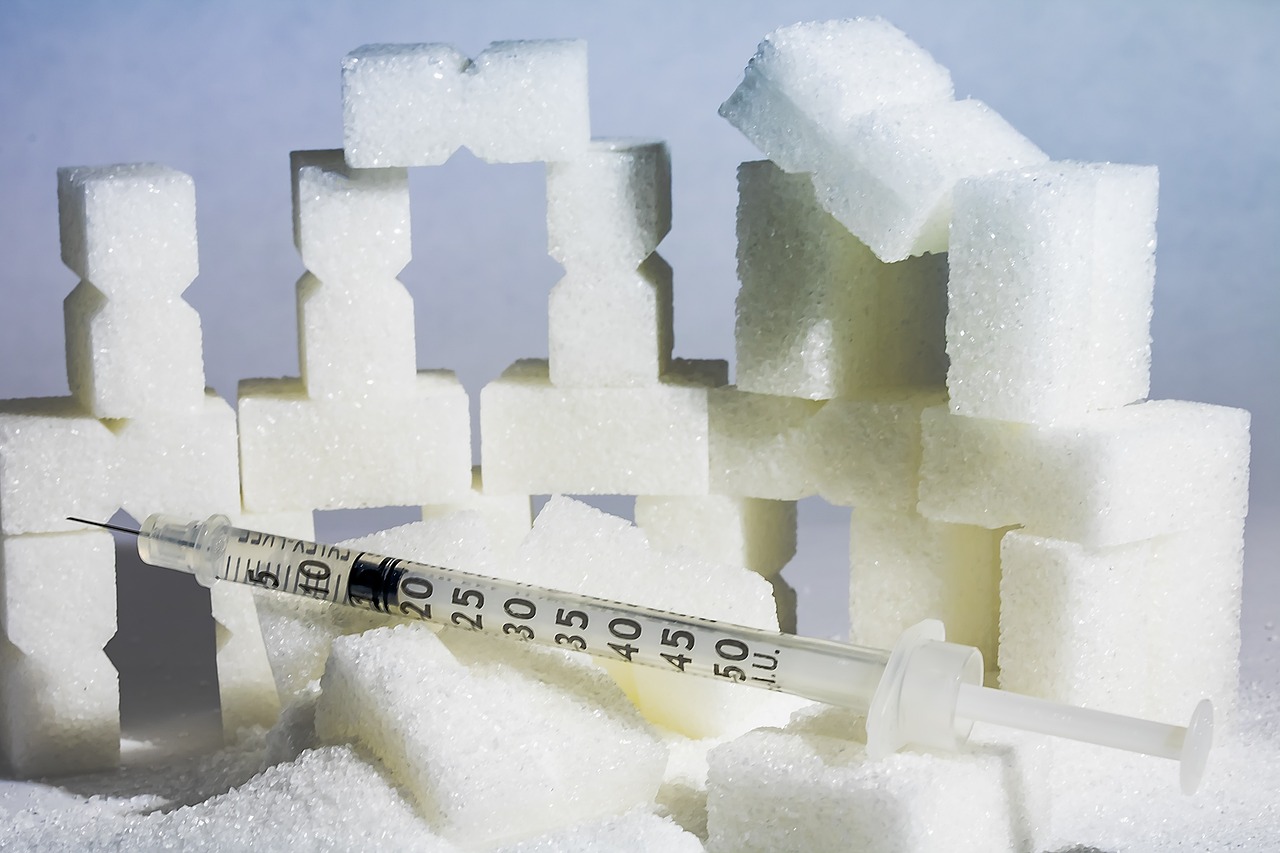
Epigenetic mechanisms participate in the development of the MetS (23). Epigenetic changes alter gene expression and cellular phenotypes without modifying the DNA sequence itself, such as via DNA methylation. This modification, the addition of a methyl group to part of the DNA molecule, prevents certain genes from being expressed (turning them “off”). Epigenetic mechanisms like this may mediate effects of environmental exposures on metabolism, as evidenced by observations of the long-term consequences of adverse intrauterine nutrition and/or gestational hyperglycaemia on the metabolic health of offspring (24). Epigenetic studies of T2D have identified differential methylation between T2D cases and controls (non-diabetic individuals) at multiple genetic loci (25-28). DNA methylation has been found to be a key regulator of lipid homeostasis and was associated with several MetS phenotypes (28).
MicroRNAs are likely to play a role in the pathogenesis of MetS (29-31). These include insulin and glucose homeostasis, cholesterol and lipid homeostasis, adipogenesis, and the inflammatory response (32-34). However, it is unclear how significant specific individual microRNAs are in the risk of developing MetS.
MetS Genetics: A Look Ahead
The search for genetic determinants of MetS has been severely challenged by the complexity of the MetS phenotype, uncertainty about the common pathogenetic mechanisms explaining the clustering of metabolic anomalies, varying definitions of MetS, and the modulating effects of lifestyle factors. Several candidate genes have been identified to regulate lipid metabolism, adiposity and insulin resistance, and have been associated with multiple MetS-related phenotypes.
However, evidence for a common genetic background explaining the clustering of metabolic traits remains limited. There is increasing evidence for the importance of epigenetic mechanisms and small regulatory RNAs, microRNAs, in the pathogenesis of MetS. Further studies in carefully phenotyped populations are needed to gain a deeper understanding of the genetic and epigenetic background of MetS.
Bibliography
- Potenza MV, Mechanick JI. The metabolic syndrome: definition, global impact, and pathophysiology. Nutr Clin Pract 2009; 24:560-77.
- National Heart, Lung and Blood Institute. What is metabolic syndrome? https://www.nhlbi.nih.gov/health/healthtopics/topics/ms Updated: June 22, 2016 Accessed September 14 2017.
- Taylor JY, Kraja AT, de las Fuentes L, Stanfill AG, Clark A, Cashion A. An overview of the genomics of metabolic syndrome. J Nurs Scholarsh. 2013;45(1):52-59.
- Fathi Dizaji B. The investigations of genetic determinants of the metabolic syndrome. Diabetes Metab Syndr. 2018;12(5):783-789.
- Wu Q, Li J, Sun X, et al. Multi-stage metabolomics and genetic analyses identified metabolite biomarkers of metabolic syndrome and their genetic determinants. EBioMedicine. 2021;74:103707.
- Rana S, Ali S, Wani HA, Mushtaq QD, Sharma S, Rehman MU. Metabolic syndrome and underlying genetic determinants-A systematic review. J Diabetes Metab Disord. 2022;21(1):1095-1104.
- Alberti KG, Eckel RH, Grundy SM, Zimmet PZ, Cleeman JI, Donato KA, Smith SC Jr. Harmonizing the metabolic syndrome: A joint interim statement of the International Diabetes Federation Task Force on Epidemiology and Prevention; National Heart, Lung, and Blood Institute; American Heart Association; World Heart Federation; International Atherosclerosis Society; and International Association for the Study of Obesity. Circulation. 2009; 120(16):1640–1645.
- Goldstein, J.; Hobbs, H.; Brown, M. The metabolic basis of inherited disease. McGraw-Hill; New York: 2001.
- Jensen HK. The molecular genetic basis and diagnosis of familial hypercholesterolemia in Denmark. Danish Medical Bulletin. 2002; 49(4):318–345.
- Hoffmann MM, Scharnagl H, Koster W, Winkler K, Wieland H, Marz W. Apolipoprotein E1 Baden (Arg(180-Cys). A new apolipoprotein E variant associated with hypertriglyceridemia. Clinica Chimica Acta. 2001; 303(1-2):41–48.
- Basson J, Simino J, Rao DC. Between candidate genes and whole genomes: Time for alternative approaches in blood pressure genetics. Current Hypertension Reports. 2012; 14(1):46–61.
- Lifton RP, Gharavi AG, Geller DS. Molecular mechanisms of human hypertension. Cell. 2001; 104(4):545–556.
- Ordovas JM, Corella D. Metabolic syndrome pathophysiology: The role of adipose tissue. Kidney International Supplement. 2008; 111:S10–S14.
- Cheung CY, Tso AW, Cheung BM, Xu A, Ong KL, Law LS, Lam KS. Genetic variants associated with persistent central obesity and the metabolic syndrome in a 12-year longitudinal study. European Journal of Endocrinology/European Federation of Endocrine Societies. 2011; 164(3):381–388.
- Glickman SG, Marn CS, Supiano MA, Dengel DR. Validity and reliability of dual-energy X-ray absorptiometry for the assessment of abdominal adiposity. Journal of Applied Physiology. 2004;97(2):509–514.
- Cashion A, Umberger R, Goodwin S, Sutter T. Collection and storage of human blood and adipose for genomic analysis of clinical sample. Research in Nursing and Health. 2011;
- Farooqi IS, O’Rahilly S. Monogenic human obesity syndromes. Recent Progress in Hormone Research. 2004; 59:409–424.
- Frayling TM, Timpson NJ, Weedon MN, Zeggini E, Freathy RM, Lindgren CM, McCarthy MI. A common variant in the FTO gene is associated with body mass index and predisposes to childhood and adult obesity. Science. 2007; 316(5826):889–894.
- Povel CM, Boer JM, Reiling E, Feskens EJ. Genetic variants and the metabolic syndrome: A systematic review. Obesity Reviews. 2011; 12(11):952–967.
- Kraja AT, Vaidya D, Pankow JS, Goodarzi MO, Assimes TL, Kullo IJ, Borecki IB. A bivariate genome-wide approach to metabolic syndrome: STAMPEED consortium. Diabetes. 2011; 60(4):1329–1339.
- Kho AN, Hayes MG, Rasmussen-Torvik L, Pacheco JA, Thompson WK, Armstrong LL, Lowe WL. Use of diverse electronic medical record systems to identify genetic risk for type 2 diabetes within a genome-wide association study. Journal of the American Medical Informatics Association. 2012;19(2):212–218.
- Delgado-Lista J, Perez-Martinez P, Garcia-Rios A, Phillips CM, Williams CM, Gulseth HL, Lopez-Miranda J. Pleiotropic effects of TCF7L2 gene variants and its modulation in the metabolic syndrome: from the LIPGENE study. Atherosclerosis. 2011; 214(1):110–116.
- Carson C, Lawson HA. Epigenetics of metabolic syndrome. Physiol Genomics. 2018 Nov 1;50(11):947-955.
- Franzago M, Fraticelli F, Stuppia L, Vitacolonna E. Nutrigenetics, epigenetics and gestational diabetes: consequences in mother and child. Epigenetics. 2019;14(3):215-235.
- Ahmed SAH, Ansari SA, Mensah-Brown EPK, Emerald BS. The role of DNA methylation in the pathogenesis of type 2 diabetes mellitus. Clin Epigenetics. 2020 Jul 11;12(1):104.
- Parveen N, Dhawan S. DNA Methylation Patterning and the Regulation of Beta Cell Homeostasis. Front Endocrinol (Lausanne). 2021 May 7;12:651258.
- Kaimala S, Kumar CA, Allouh MZ, Ansari SA, Emerald BS. Epigenetic modifications in pancreas development, diabetes, and therapeutics. Med Res Rev. 2022 May;42(3):1343-1371.
- Samblas M, Milagro FI, Martínez A. DNA methylation markers in obesity, metabolic syndrome, and weight loss. Epigenetics. 2019 May;14(5):421-444.
- Heneghan HM, Miller N, Kerin MJ. Role of microRNAs in obesity and the metabolic syndrome. Obes Rev. 2010;11(5):354-361.
- Solís-Toro D, Mosquera Escudero M, García-Perdomo HA. Association between circulating microRNAs and the metabolic syndrome in adult populations: A systematic review. Diabetes Metab Syndr. 2022;16(1):102376.
- Lee W. MicroRNA, Insulin Resistance, and Metabolic Disorders. Int J Mol Sci. 2022;23(24):16215.
- Dalgaard LT, Sørensen AE, Hardikar AA, Joglekar MV. The microRNA-29 family: role in metabolism and metabolic disease. Am J Physiol Cell Physiol. 2022;323(2):C367-C377.
- Zhen YF, Zhang YJ, Zhao H, Ma HJ, Song GY. MicroRNA-802 regulates hepatic insulin sensitivity and glucose metabolism. Int J Clin Exp Pathol. 2018;11(5):2440-2449.
- Włodarski A, Strycharz J, Wróblewski A, Kasznicki J, Drzewoski J, Śliwińska A. The Role of microRNAs in Metabolic Syndrome-Related Oxidative Stress. Int J Mol Sci. 2020;21(18):6902.
Picture Credit:
Picture of blood pressure measurement, “KRT LIFE HEALTH-BLOOD-PRESSURE PG“, by Fort George G. Meade Public Affairs Office. CC BY 2.0 Attribution 2.0 Generic Deed.